Abstract
Neuroinflammation is characterized by reactive microglia and astrocytes (collectively called gliosis) in the central nervous system and is considered as one of the main pathological hallmarks in different neurodegenerative diseases such as Alzheimer’s disease, age-related dementia, and multiple sclerosis. Upon activation, glia undergoes structural and morphological changes such as the microglial cells swell in size and astrocytes become bushy, which play both beneficial and detrimental roles. Hence, they are unable to perform the normal physiological role in brain immunity. Curcumin, a cytokine suppressive anti-inflammatory drug, has a high proven pre-clinical potency and efficacy to reverse chronic neuroinflammation by attenuating the activation and morphological changes that occur in the microglia and astrocytes. This review will highlight the recent findings on the tree structure changes of microglia and astrocytes in neuroinflammation and the effects of curcumin against the activation and morphology of glial cells.
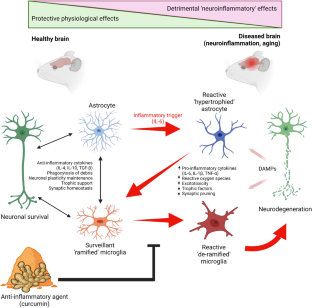
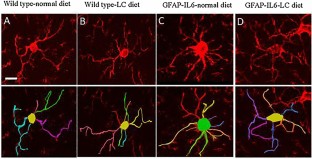
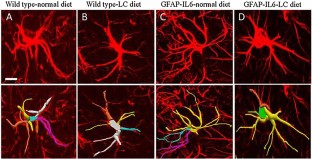
Similar content being viewed by others
References
Gamage R et al (2020) Cholinergic modulation of glial function during aging and chronic neuroinflammation. Front Cell Neurosci. https://doi.org/10.3389/fncel.2020.577912
Norden DM, Muccigrosso MM, Godbout JP (2015) Microglial priming and enhanced reactivity to secondary insult in aging, and traumatic CNS injury, and neurodegenerative disease. Neuropharmacology 96(Pt A):29–41
Godbout JP et al (2005) Exaggerated neuroinflammation and sickness behavior in aged mice following activation of the peripheral innate immune system. FASEB J 19(10):1329–1331
Lee EG et al (2000) Failure to regulate TNF-induced NF-kappaB and cell death responses in A20-deficient mice. Science 289(5488):2350–2354
Cerbai F et al (2012) The neuron-astrocyte-microglia triad in normal brain ageing and in a model of neuroinflammation in the rat hippocampus. PLoS ONE 7(9):e45250
Henry CJ et al (2009) Peripheral lipopolysaccharide (LPS) challenge promotes microglial hyperactivity in aged mice that is associated with exaggerated induction of both pro-inflammatory IL-1beta and anti-inflammatory IL-10 cytokines. Brain Behav Immun 23(3):309–317
Gavilán MP et al (2007) Molecular and cellular characterization of the age-related neuroinflammatory processes occurring in normal rat hippocampus: potential relation with the loss of somatostatin GABAergic neurons. J Neurochem 103(3):984–996
Sparkman NL et al (2006) Interleukin-6 facilitates lipopolysaccharide-induced disruption in working memory and expression of other proinflammatory cytokines in hippocampal neuronal cell layers. J Neurosci 26(42):10709–10716
Ye SM, Johnson RW (2001) An age-related decline in interleukin-10 may contribute to the increased expression of interleukin-6 in brain of aged mice. Neuroimmunomodulation 9(4):183–192
Maher FO, Nolan Y, Lynch MA (2005) Downregulation of IL-4-induced signalling in hippocampus contributes to deficits in LTP in the aged rat. Neurobiol Aging 26(5):717–728
Chen JL, Penhune VB, Zatorre RJ (2008) Listening to musical rhythms recruits motor regions of the brain. Cereb Cortex 18(12):2844–2854
Streit WJ, Mrak RE, Griffin WST (2004) Microglia and neuroinflammation: a pathological perspective. J Neuroinflamm 1(1):14
Sen MK et al (2020) Revisiting the pathoetiology of multiple sclerosis: has the tail been wagging the mouse? Front Immunol 11:572186
Xie A et al (2014) Shared mechanisms of neurodegeneration in Alzheimer’s disease and Parkinson’s disease. Biomed Res Int 2014:648740
Kettenmann H, Kirchhoff F, Verkhratsky A (2013) Microglia: new roles for the synaptic stripper. Neuron 77(1):10–18
Colonna M, Butovsky O (2017) Microglia function in the central nervous system during health and neurodegeneration. Annu Rev Immunol 35:441–468
Wolf SA, Boddeke H, Kettenmann H (2017) Microglia in physiology and disease. Annu Rev Physiol 79:619–643
Kozai TD et al (2012) In vivo two-photon microscopy reveals immediate microglial reaction to implantation of microelectrode through extension of processes. J Neural Eng 9(6):066001
Nimmerjahn A, Kirchhoff F, Helmchen F (2005) Resting microglial cells are highly dynamic surveillants of brain parenchyma in vivo. Science 308(5726):1314–1318
Lawson LJ et al (1990) Heterogeneity in the distribution and morphology of microglia in the normal adult mouse brain. Neuroscience 39(1):151–170
Gomez-Nicola D, Perry VH (2015) Microglial dynamics and role in the healthy and diseased brain: a paradigm of functional plasticity. Neuroscientist 21(2):169–184
Vinet J et al (2012) Neuroprotective function for ramified microglia in hippocampal excitotoxicity. J Neuroinflamm 9:27
Hinwood M et al (2013) Chronic stress induced remodeling of the prefrontal cortex: structural re-organization of microglia and the inhibitory effect of minocycline. Cereb Cortex 23(8):1784–1797
Heindl S et al (2018) Automated morphological analysis of microglia after stroke. Front Cell Neurosci 12:106
Hovens IB et al (2014) Postoperative cognitive dysfunction: involvement of neuroinflammation and neuronal functioning. Brain Behav Immun 38:202–210
Ohsawa K et al (2004) Microglia/macrophage-specific protein Iba1 binds to fimbrin and enhances its actin-bundling activity. J Neurochem 88(4):844–856
VanGuilder HD et al (2011) Concurrent hippocampal induction of MHC II pathway components and glial activation with advanced aging is not correlated with cognitive impairment. J Neuroinflamm 8:138
Torres-Platas SG et al (2014) Morphometric characterization of microglial phenotypes in human cerebral cortex. J Neuroinflamm 11:12
Heneka MT et al (2015) Neuroinflammation in Alzheimer’s disease. Lancet Neurol 14(4):388–405
Karperien A, Ahammer H, Jelinek HF (2013) Quantitating the subtleties of microglial morphology with fractal analysis. Front Cell Neurosci 7:3
Ransohoff RM (2016) A polarizing question: do M1 and M2 microglia exist? Nat Neurosci 19(8):987–991
Otsu N (1979) A threshold selection method from gray-level histograms. IEEE Trans Syst Man Cybern 9(1):62–66
Abdolhoseini M et al (2019) Segmentation, tracing, and quantification of microglial cells from 3D image stacks. Sci Rep 9(1):8557
Clarke LE, Barres BA (2013) Emerging roles of astrocytes in neural circuit development. Nat Rev Neurosci 14(5):311–321
Voskuhl RR et al (2009) Reactive astrocytes form scar-like perivascular barriers to leukocytes during adaptive immune inflammation of the CNS. J Neurosci 29(37):11511–11522
Mayo L, Quintana FJ, Weiner HL (2012) The innate immune system in demyelinating disease. Immunol Rev 248(1):170–187
Wilhelmsson U et al (2006) Redefining the concept of reactive astrocytes as cells that remain within their unique domains upon reaction to injury. Proc Natl Acad Sci USA 103(46):17513–17518
Sofroniew MV (2009) Molecular dissection of reactive astrogliosis and glial scar formation. Trends Neurosci 32(12):638–647
Hara M et al (2017) Interaction of reactive astrocytes with type I collagen induces astrocytic scar formation through the integrin-N-cadherin pathway after spinal cord injury. Nat Med 23(7):818–828
Mrak RE, Griffin WS (2005) Glia and their cytokines in progression of neurodegeneration. Neurobiol Aging 26(3):349–354
Ahmad MH, Fatima M, Mondal AC (2019) Influence of microglia and astrocyte activation in the neuroinflammatory pathogenesis of Alzheimer’s disease: rational insights for the therapeutic approaches. J Clin Neurosci 59:6–11
Liddelow SA et al (2017) Neurotoxic reactive astrocytes are induced by activated microglia. Nature 541(7638):481–487
Chandran B, Goel A (2012) A randomized, pilot study to assess the efficacy and safety of curcumin in patients with active rheumatoid arthritis. Phytother Res 26(11):1719–1725
Sanmukhani J et al (2014) Efficacy and safety of curcumin in major depressive disorder: a randomized controlled trial. Phytother Res 28(4):579–585
Rao CV, Simi B, Reddy BS (1993) Inhibition by dietary curcumin of azoxymethane-induced ornithine decarboxylase, tyrosine protein kinase, arachidonic acid metabolism and aberrant crypt foci formation in the rat colon. Carcinogenesis 14(11):2219–2225
Sundaram JR et al (2017) Curcumin ameliorates neuroinflammation, neurodegeneration, and memory deficits in p25 transgenic mouse model that bears hallmarks of Alzheimer’s disease. J Alzheimer’s Dis 60(4):1429–1442
Parada E et al (2015) Microglial HO-1 induction by curcumin provides antioxidant, antineuroinflammatory, and glioprotective effects. Mol Nutr Food Res 59(9):1690–1700
Ji FT et al (2013) Curcumin exerts antinociceptive effects by inhibiting the activation of astrocytes in spinal dorsal horn and the intracellular extracellular signal-regulated kinase signaling pathway in rat model of chronic constriction injury. Chin Med J (Engl) 126(6):1125–1131
Ralay Ranaivo H et al (2006) Glia as a therapeutic target: selective suppression of human amyloid-beta-induced upregulation of brain proinflammatory cytokine production attenuates neurodegeneration. J Neurosci 26(2):662–670
Vela JM et al (1995) Morphology and distribution of microglial cells in the young and adult mouse cerebellum. J Comp Neurol 361(4):602–616
Yamada J, Jinno S (2013) Novel objective classification of reactive microglia following hypoglossal axotomy using hierarchical cluster analysis. J Comp Neurol 521(5):1184–1201
Leng F, Edison P (2021) Neuroinflammation and microglial activation in Alzheimer disease: where do we go from here? Nat Rev Neurol 17(3):157–172
Sparkman NL, Johnson RW (2008) Neuroinflammation associated with aging sensitizes the brain to the effects of infection or stress. Neuroimmunomodulation 15(4–6):323–330
Perry VH, Matyszak MK, Fearn S (1993) Altered antigen expression of microglia in the aged rodent CNS. Glia 7(1):60–67
Sheng JG, Mrak RE, Griffin WS (1998) Enlarged and phagocytic, but not primed, interleukin-1 alpha-immunoreactive microglia increase with age in normal human brain. Acta Neuropathol 95(3):229–234
Streit WJ et al (2004) Dystrophic microglia in the aging human brain. Glia 45(2):208–212
Perry VH, Newman TA, Cunningham C (2003) The impact of systemic infection on the progression of neurodegenerative disease. Nat Rev Neurosci 4(2):103–112
Streit WJ et al (2009) Dystrophic (senescent) rather than activated microglial cells are associated with tau pathology and likely precede neurodegeneration in Alzheimer’s disease. Acta Neuropathol 118(4):475–485
Choi JH et al (2007) Age-related changes in ionized calcium-binding adapter molecule 1 immunoreactivity and protein level in the gerbil hippocampal CA1 region. J Vet Med Sci 69(11):1131–1136
Hwang IK et al (2008) Comparison of ionized calcium-binding adapter molecule 1 immunoreactivity of the hippocampal dentate gyrus and CA1 region in adult and aged dogs. Neurochem Res 33(7):1309–1315
Schuitemaker A et al (2012) Microglial activation in healthy aging. Neurobiol Aging 33(6):1067–1072
Lull ME, Block ML (2010) Microglial activation and chronic neurodegeneration. Neurotherapeutics 7(4):354–365
Kreutzberg GW (1996) Microglia: a sensor for pathological events in the CNS. Trends Neurosci 19(8):312–318
Bedi SS et al (2018) Therapeutic time window of multipotent adult progenitor therapy after traumatic brain injury. J Neuroinflamm 15(1):84
Morrison H et al (2017) Quantitative microglia analyses reveal diverse morphologic responses in the rat cortex after diffuse brain injury. Sci Rep 7(1):13211
Davis EJ, Foster TD, Thomas WE (1994) Cellular forms and functions of brain microglia. Brain Res Bull 34(1):73–78
Ullah F et al (2020) Effects of a solid lipid curcumin particle formulation on chronic activation of microglia and astroglia in the GFAP-IL6 mouse model. Sci Rep 10(1):2365
Ullah F et al (2020) Evaluation of phytosomal curcumin as an anti-inflammatory agent for chronic glial activation in the GFAP-IL6 mouse model. Front Neurosci. https://doi.org/10.3389/fnins.2020.00170
Plescher M et al (2018) Plaque-dependent morphological and electrophysiological heterogeneity of microglia in an Alzheimer’s disease mouse model. Glia 66(7):1464–1480
Franco-Bocanegra DK et al (2021) Microglial morphology in Alzheimer’s disease and after Aβ immunotherapy. Sci Rep 11(1):15955
Sochocka M, Diniz BS, Leszek J (2017) Inflammatory response in the CNS: friend or foe? Mol Neurobiol 54(10):8071–8089
Kabba JA et al (2018) Microglia: housekeeper of the central nervous system. Cell Mol Neurobiol 38(1):53–71
Bushong EA, Martone ME, Ellisman MH (2004) Maturation of astrocyte morphology and the establishment of astrocyte domains during postnatal hippocampal development. Int J Dev Neurosci 22(2):73–86
Miller RH, Raff MC (1984) Fibrous and protoplasmic astrocytes are biochemically and developmentally distinct. J Neurosci 4(2):585–592
Henneberger C et al (2010) Long-term potentiation depends on release of d-serine from astrocytes. Nature 463(7278):232–236
Uwechue NM et al (2012) Activation of glutamate transport evokes rapid glutamine release from perisynaptic astrocytes. J Physiol 590(10):2317–2331
Takano T et al (2006) Astrocyte-mediated control of cerebral blood flow. Nat Neurosci 9(2):260–267
Marín-Padilla M (1995) Prenatal development of fibrous (white matter), protoplasmic (gray matter), and layer I astrocytes in the human cerebral cortex: a Golgi study. J Comp Neurol 357(4):554–572
Stone DJ et al (1998) Increased synaptic sprouting in response to estrogen via an apolipoprotein E-dependent mechanism: implications for Alzheimer’s disease. J Neurosci 18(9):3180–3185
Sofroniew MV, Vinters HV (2010) Astrocytes: biology and pathology. Acta Neuropathol 119(1):7–35
Gorina R et al (2011) Astrocyte TLR4 activation induces a proinflammatory environment through the interplay between MyD88-dependent NFκB signaling, MAPK, and Jak1/Stat1 pathways. Glia 59(2):242–255
Paintlia AS et al (2013) Modulation of Rho-Rock signaling pathway protects oligodendrocytes against cytokine toxicity via PPAR-α-dependent mechanism. Glia 61(9):1500–1517
Villapol S, Byrnes KR, Symes AJ (2014) Temporal dynamics of cerebral blood flow, cortical damage, apoptosis, astrocyte-vasculature interaction and astrogliosis in the pericontusional region after traumatic brain injury. Front Neurol 5:82
Liu Z et al (2014) Beneficial effects of gfap/vimentin reactive astrocytes for axonal remodeling and motor behavioral recovery in mice after stroke. Glia 62(12):2022–2033
Li H et al (2014) Histological, cellular and behavioral assessments of stroke outcomes after photothrombosis-induced ischemia in adult mice. BMC Neurosci 15:58
Stapf C et al (2002) Concurrent arterial aneurysms in brain arteriovenous malformations with haemorrhagic presentation. J Neurol Neurosurg Psychiatry 73(3):294–298
Mestriner RG et al (2015) Astrocyte morphology after ischemic and hemorrhagic experimental stroke has no influence on the different recovery patterns. Behav Brain Res 278:257–261
Oberheim NA et al (2009) Uniquely hominid features of adult human astrocytes. J Neurosci 29(10):3276–3287
Torres-Platas SG et al (2011) Astrocytic hypertrophy in anterior cingulate white matter of depressed suicides. Neuropsychopharmacology 36(13):2650–2658
Miller AH, Maletic V, Raison CL (2009) Inflammation and its discontents: the role of cytokines in the pathophysiology of major depression. Biol Psychiatry 65(9):732–741
Aggarwal BB, Kumar A, Bharti AC (2003) Anticancer potential of curcumin: preclinical and clinical studies. Anticancer Res 23(1/A):363–398
Ullah F et al (2017) High bioavailability curcumin: an anti-inflammatory and neurosupportive bioactive nutrient for neurodegenerative diseases characterized by chronic neuroinflammation. Arch Toxicol 91(4):1623–1634
Amalraj A et al (2017) Biological activities of curcuminoids, other biomolecules from turmeric and their derivatives—a review. J Tradit Complement Med 7(2):205–233
Farkhondeh T et al (2019) The impact of curcumin and its modified formulations on Alzheimer’s disease. J Cell Physiol 234(10):16953–16965
Panaro MA et al (2020) The emerging role of curcumin in the modulation of TLR-4 signaling pathway: focus on neuroprotective and anti-rheumatic properties. Int J Mol Sci 21(7):2299
Johnson GB, Brunn GJ, Platt JL (2003) Activation of mammalian Toll-like receptors by endogenous agonists. Crit Rev Immunol 23(1–2):15–44
Kigerl KA et al (2007) Toll-like receptor (TLR)-2 and TLR-4 regulate inflammation, gliosis, and myelin sparing after spinal cord injury. J Neurochem 102(1):37–50
Takeda K, Akira S (2004) TLR signaling pathways. Semin Immunol 16(1):3–9
Ni H et al (2015) Curcumin modulates TLR4/NF-κB inflammatory signaling pathway following traumatic spinal cord injury in rats. J Spinal Cord Med 38(2):199–206
Bharti AC, Donato N, Aggarwal BB (2003) Curcumin (diferuloylmethane) inhibits constitutive and IL-6-inducible STAT3 phosphorylation in human multiple myeloma cells. J Immunol 171(7):3863–3871
Kim HY et al (2003) Curcumin suppresses Janus kinase-STAT inflammatory signaling through activation of Src homology 2 domain-containing tyrosine phosphatase 2 in brain microglia. J Immunol 171(11):6072–6079
Ray B, Lahiri DK (2009) Neuroinflammation in Alzheimer’s disease: different molecular targets and potential therapeutic agents including curcumin. Curr Opin Pharmacol 9(4):434–444
Kodali M et al (2018) Curcumin treatment leads to better cognitive and mood function in a model of Gulf War Illness with enhanced neurogenesis, and alleviation of inflammation and mitochondrial dysfunction in the hippocampus. Brain Behav Immun 69:499–514
Burgos-Morón E, López-Lázaro M et al (2010) The dark side of curcumin. Int J Cancer 126:177
Ghasemi F et al (2019) Effects of curcumin on microglial cells. Neurotox Res 36(1):12–26
Chin D et al (2013) Neuroprotective properties of curcumin in Alzheimer’s disease—merits and limitations. Curr Med Chem 20(32):3955–3985
Jamwal R (2018) Bioavailable curcumin formulations: a review of pharmacokinetic studies in healthy volunteers. J Integr Med 16(6):367–374
Antony B et al (2008) A pilot cross-over study to evaluate human oral bioavailability of BCM-95CG (Biocurcumax), a novel bioenhanced preparation of curcumin. Indian J Pharm Sci 70(4):445–449
Stohs SJ et al (2020) Highly bioavailable forms of curcumin and promising avenues for curcumin-based research and application: a review. Molecules 25(6):1397
Cuomo J et al (2011) Comparative absorption of a standardized curcuminoid mixture and its lecithin formulation. J Nat Prod 74(4):664–669
Gota VS et al (2010) Safety and pharmacokinetics of a solid lipid curcumin particle formulation in osteosarcoma patients and healthy volunteers. J Agric Food Chem 58(4):2095–2099
Jäger R et al (2014) Comparative absorption of curcumin formulations. Nutr J 13:11
Klickovic U et al (2014) Human pharmacokinetics of high dose oral curcumin and its effect on heme oxygenase-1 expression in healthy male subjects. Biomed Res Int 2014:458592
Shoba G et al (1998) Influence of piperine on the pharmacokinetics of curcumin in animals and human volunteers. Planta Med 64(4):353–356
Purpura M et al (2018) Analysis of different innovative formulations of curcumin for improved relative oral bioavailability in human subjects. Eur J Nutr 57(3):929–938
Gopi S et al (2017) Comparative oral absorption of curcumin in a natural turmeric matrix with two other curcumin formulations: an open-label parallel-arm study. Phytother Res 31(12):1883–1891
Kumar D et al (2016) Enhanced bioavailability and relative distribution of free (unconjugated) curcuminoids following the oral administration of a food-grade formulation with fenugreek dietary fibre: a randomised double-blind crossover study. J Funct Foods 22:578–587
Sasaki H et al (2011) Innovative preparation of curcumin for improved oral bioavailability. Biol Pharm Bull 34(5):660–665
Kanai M et al (2012) Dose-escalation and pharmacokinetic study of nanoparticle curcumin, a potential anticancer agent with improved bioavailability, in healthy human volunteers. Cancer Chemother Pharmacol 69(1):65–70
Madhavi D, Kagan D (2014) Bioavailability of a sustained release formulation of curcumin. Integr Med (Encinitas) 13(3):24–30
Briskey D et al (2019) Increased bioavailability of curcumin using a novel dispersion technology system (LipiSperse®). Eur J Nutr 58(5):2087–2097
Gyengesi E et al (2018) Investigation into the effects of tenilsetam on markers of neuroinflammation in GFAP-IL6 mice. Pharm Res 35(1):22
Campbell IL et al (1993) Neurologic disease induced in transgenic mice by cerebral overexpression of interleukin 6. Proc Natl Acad Sci USA 90(21):10061–10065
Maiti P, Paladugu L, Dunbar GL (2018) Solid lipid curcumin particles provide greater anti-amyloid, anti-inflammatory and neuroprotective effects than curcumin in the 5xFAD mouse model of Alzheimer’s disease. BMC Neurosci 19(1):7
Acknowledgements
Not applicable.
Funding
No special funding was received for this work.
Author information
Authors and Affiliations
Contributions
FU designed, drafted, and wrote the manuscript. RG wrote sections, edited and reviewed the manuscript and prepared Fig 1. MKS reviewed and edited the manuscript. EG supervised, advised, edited and reviewed the manuscript. All authors approved the final version of the manuscript.
Corresponding author
Ethics declarations
Conflict of interest
The authors declare no competing financial interests.
Additional information
Publisher's Note
Springer Nature remains neutral with regard to jurisdictional claims in published maps and institutional affiliations.
Rights and permissions
About this article
Cite this article
Ullah, F., Gamage, R., Sen, M.K. et al. The Effects of Modified Curcumin Preparations on Glial Morphology in Aging and Neuroinflammation. Neurochem Res 47, 813–824 (2022). https://doi.org/10.1007/s11064-021-03499-4
Received:
Revised:
Accepted:
Published:
Issue Date:
DOI: https://doi.org/10.1007/s11064-021-03499-4