Abstract
Many mechanisms underlying an effective immunotherapy-induced antitumour response are transient and critically time dependent. This is equally true for several immunological events in the tumour microenvironment induced by other cancer treatments. Immune checkpoint therapy (ICT) has proven to be very effective in the treatment of some cancers, but unfortunately, with many cancer types, most patients do not experience a benefit. To improve outcomes, a multitude of clinical trials are testing combinations of ICT with various other treatment modalities. Ideally, those combination treatments should take time-dependent immunological events into account. Recent studies have started to map the dynamic cellular and molecular changes that occur during treatment with ICT, in the tumour and systemically. Here, we overlay the dynamic ICT response with the therapeutic response following surgery, radiotherapy, chemotherapy and targeted therapies. We propose that by combining treatments in a time-conscious manner, we may optimally exploit the interactions between the individual therapies.
This is a preview of subscription content, access via your institution
Access options
Access Nature and 54 other Nature Portfolio journals
Get Nature+, our best-value online-access subscription
$29.99 / 30 days
cancel any time
Subscribe to this journal
Receive 12 print issues and online access
$209.00 per year
only $17.42 per issue
Buy this article
- Purchase on Springer Link
- Instant access to full article PDF
Prices may be subject to local taxes which are calculated during checkout
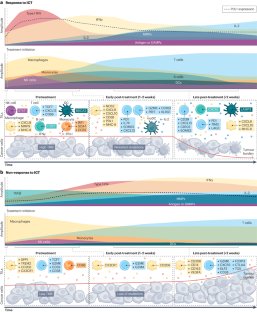
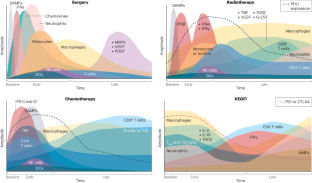
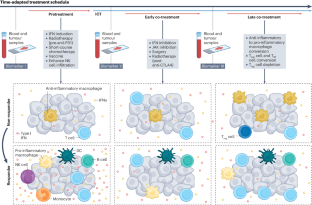
Similar content being viewed by others
References
Zemek, R. M. et al. Sensitizing the tumor microenvironment to immune checkpoint therapy. Front. Immunol. 11, 223 (2020).
Lesterhuis, W. J. et al. Dynamic versus static biomarkers in cancer immune checkpoint blockade: unravelling complexity. Nat. Rev. Drug Discov. 16, 264–272 (2017).
Germain, R. N. The art of the probable: system control in the adaptive immune system. Science 293, 240–245 (2001).
Zemek, R. M. et al. Bilateral murine tumor models for characterizing the response to immune checkpoint blockade. Nat. Protoc. 15, 1628–1648 (2020).
Combes, A. J., Samad, B. & Krummel, M. F. Defining and using immune archetypes to classify and treat cancer. Nat. Rev. Cancer 23, 491–505 (2023).
Edwards, J. et al. CD103+ tumor-resident CD8+ T cells are associated with improved survival in immunotherapy-naïve melanoma patients and expand significantly during anti-PD-1 treatment. Clin. Cancer Res. 24, 3036–3045 (2018).
Gide, T. N. et al. Distinct immune cell populations define response to anti-PD-1 monotherapy and anti-PD-1/anti-CTLA-4 combined therapy. Cancer Cell 35, 238–255.e6 (2019).
Zhang, Y. et al. Single-cell analyses reveal key immune cell subsets associated with response to PD-L1 blockade in triple-negative breast cancer. Cancer Cell 39, 1578–1593.e8 (2021).
Liu, B., Zhang, Y., Wang, D., Hu, X. & Zhang, Z. Single-cell meta-analyses reveal responses of tumor-reactive CXCL13+ T cells to immune-checkpoint blockade. Nat. Cancer 3, 1123–1136 (2022).
Magen, A. et al. Intratumoral dendritic cell-CD4+ T helper cell niches enable CD8+ T cell differentiation following PD-1 blockade in hepatocellular carcinoma. Nat. Med. 29, 1389–1399 (2023).
Bassez, A. et al. A single-cell map of intratumoral changes during anti-PD1 treatment of patients with breast cancer. Nat. Med. 27, 820–832 (2021).
Hollern, D. P. et al. B cells and T follicular helper cells mediate response to checkpoint inhibitors in high mutation burden mouse models of breast cancer. Cell 179, 1191–1206.e21 (2019).
Riaz, N. et al. Tumor and microenvironment evolution during immunotherapy with nivolumab. Cell 171, 934–949.e16 (2017).
Chen, I. X. et al. A bilateral tumor model identifies transcriptional programs associated with patient response to immune checkpoint blockade. Proc. Natl Acad. Sci. USA 117, 23684–23694 (2020).
Carroll, T. M. et al. Tumor monocyte content predicts immunochemotherapy outcomes in esophageal adenocarcinoma. Cancer Cell 41, 1222–1241.e7 (2023).
Jaiswal, A. et al. An activation to memory differentiation trajectory of tumor-infiltrating lymphocytes informs metastatic melanoma outcomes. Cancer Cell 40, 524–544.e5 (2022).
Zheng, L. et al. Pan-cancer single-cell landscape of tumor-infiltrating T cells. Science 374, abe6474 (2021).
Verma, V. et al. PD-1 blockade in subprimed CD8 cells induces dysfunctional PD-1+CD38hi cells and anti-PD-1 resistance. Nat. Immunol. 20, 1231–1243 (2019).
Vilain, R. E. et al. Dynamic changes in PD-L1 expression and immune infiltrates early during treatment predict response to PD-1 blockade in melanoma. Clin. Cancer Res. 23, 5024–5033 (2017).
Chen, P. L. et al. Analysis of immune signatures in longitudinal tumor samples yields insight into biomarkers of response and mechanisms of resistance to immune checkpoint blockade. Cancer Discov. 6, 827–837 (2016).
Qu, Y. et al. Baseline frequency of inflammatory CXCL9-expressing tumor-associated macrophages predicts response to avelumab treatment. Cell Rep. 32, 107873 (2020).
Kersten, K. et al. Spatiotemporal co-dependency between macrophages and exhausted CD8+ T cells in cancer. Cancer Cell 40, 624–638.e9 (2022).
Broz, M. L. et al. Dissecting the tumor myeloid compartment reveals rare activating antigen-presenting cells critical for T cell immunity. Cancer Cell 26, 638–652 (2014).
Salmon, H. et al. Expansion and activation of CD103+ dendritic cell progenitors at the tumor site enhances tumor responses to therapeutic PD-L1 and BRAF inhibition. Immunity 44, 924–938 (2016).
Mayoux, M. et al. Dendritic cells dictate responses to PD-L1 blockade cancer immunotherapy. Sci. Transl. Med. 12, eaav7431 (2020).
Anagnostou, V. et al. Integrative tumor and immune cell multi-omic analyses predict response to immune checkpoint blockade in melanoma. Cell Rep. Med. 1, 100139 (2020).
Helmink, B. A. et al. B cells and tertiary lymphoid structures promote immunotherapy response. Nature 577, 549–555 (2020).
Petitprez, F. et al. B cells are associated with survival and immunotherapy response in sarcoma. Nature 577, 556–560 (2020).
Vanhersecke, L. et al. Mature tertiary lymphoid structures predict immune checkpoint inhibitor efficacy in solid tumors independently of PD-L1 expression. Nat. Cancer 2, 794–802 (2021).
Wise-Draper, T. M. et al. Phase II clinical trial of neoadjuvant and adjuvant pembrolizumab in resectable local–regionally advanced head and neck squamous cell carcinoma. Clin. Cancer Res. 28, 1345–1352 (2022).
Grasso, C. S. et al. Conserved interferon-γ signaling drives clinical response to immune checkpoint blockade therapy in melanoma. Cancer Cell 38, 500–515.e3 (2020).
Zemek, R. M. et al. Sensitization to immune checkpoint blockade through activation of a STAT1/NK axis in the tumor microenvironment. Sci. Transl. Med. 11, eaav7816 (2019).
Bald, T. et al. Immune cell–poor melanomas benefit from PD-1 blockade after targeted type I IFN activation. Cancer Discov. 4, 674–687 (2014).
Barry, K. C. et al. A natural killer–dendritic cell axis defines checkpoint therapy–responsive tumor microenvironments. Nat. Med. 24, 1178–1191 (2018).
Zemek, R. M. et al. Temporally restricted activation of IFNβ signaling underlies response to immune checkpoint therapy in mice. Nat. Commun. 13, 4895 (2022).
Wu, T. et al. The TCF1-Bcl6 axis counteracts type I interferon to repress exhaustion and maintain T cell stemness. Sci. Immunol. 1, eaai8593 (2016).
Benci, J. L. et al. Opposing functions of interferon coordinate adaptive and innate immune responses to cancer immune checkpoint blockade. Cell 178, 933–948.e14 (2019).
Gubin, M. M. et al. High-dimensional analysis delineates myeloid and lymphoid compartment remodeling during successful immune-checkpoint cancer therapy. Cell 175, 1014–1030.e19 (2018).
Kwart, D. et al. Cancer cell-derived type I interferons instruct tumor monocyte polarization. Cell Rep. 41, 111769 (2022).
Schetters, S. T. et al. Monocyte-derived APCs are central to the response of PD1 checkpoint blockade and provide a therapeutic target for combination therapy. J. Immunother. Cancer 8, e000588 (2020).
Miller, B. C. et al. Subsets of exhausted CD8+ T cells differentially mediate tumor control and respond to checkpoint blockade. Nat. Immunol. 20, 326–336 (2019).
Siddiqui, I. et al. Intratumoral Tcf1+PD-1+CD8+ T cells with stem-like properties promote tumor control in response to vaccination and checkpoint blockade immunotherapy. Immunity 50, 195–211.e10 (2019).
Kallies, A., Zehn, D. & Utzschneider, D. T. Precursor exhausted T cells: key to successful immunotherapy? Nat. Rev. Immunol. 20, 128–136 (2020).
Gebhardt, T., Park, S. L. & Parish, I. A. Stem-like exhausted and memory CD8+ T cells in cancer. Nat. Rev. Cancer 23, 780–798 (2023).
Simoni, Y. et al. Bystander CD8+ T cells are abundant and phenotypically distinct in human tumour infiltrates. Nature 557, 575–579 (2018).
Giles, J. R. et al. Shared and distinct biological circuits in effector, memory and exhausted CD8+ T cells revealed by temporal single-cell transcriptomics and epigenetics. Nat. Immunol. 23, 1600–1613 (2022).
Taylor, M. A. et al. Longitudinal immune characterization of syngeneic tumor models to enable model selection for immune oncology drug discovery. J. Immunother. Cancer 7, 328 (2019).
Principe, N. et al. Tumor infiltrating effector memory antigen-specific CD8+ T cells predict response to immune checkpoint therapy. Front. Immunol. 11, 584423 (2020).
Kidman, J. et al. Immune checkpoint therapy responders display early clonal expansion of tumor infiltrating lymphocytes. Oncoimmunology 13, 2345859 (2024).
Kruse, B. et al. CD4+ T cell-induced inflammatory cell death controls immune-evasive tumours. Nature 618, 1033–1040 (2023).
Sade-Feldman, M. et al. Defining T cell states associated with response to checkpoint immunotherapy in melanoma. Cell 175, 998–1013.e20 (2018).
Voorwerk, L. et al. Immune induction strategies in metastatic triple-negative breast cancer to enhance the sensitivity to PD-1 blockade: the TONIC trial. Nat. Med. 25, 920–928 (2019).
Shiao, S. L. et al. Single-cell and spatial profiling identify three response trajectories to pembrolizumab and radiation therapy in triple negative breast cancer. Cancer Cell 42, 70–84.e8 (2024).
Amaria, R. N. et al. Neoadjuvant immune checkpoint blockade in high-risk resectable melanoma. Nat. Med. 24, 1649–1654 (2018).
Roh, W. et al. Integrated molecular analysis of tumor biopsies on sequential CTLA-4 and PD-1 blockade reveals markers of response and resistance. Sci. Transl. Med. 9, eaah3560 (2017).
Luoma, A. M. et al. Tissue-resident memory and circulating T cells are early responders to pre-surgical cancer immunotherapy. Cell 185, 2918–2935.e29 (2022).
Yost, K. E. et al. Clonal replacement of tumor-specific T cells following PD-1 blockade. Nat. Med. 25, 1251–1259 (2019).
Caushi, J. X. et al. Transcriptional programs of neoantigen-specific TIL in anti-PD-1-treated lung cancers. Nature 596, 126–132 (2021).
Oliveira, G. & Wu, C. J. Dynamics and specificities of T cells in cancer immunotherapy. Nat. Rev. Cancer 23, 295–316 (2023).
Cachot, A. et al. Tumor-specific cytolytic CD4 T cells mediate immunity against human cancer. Sci. Adv. 7, eabe3348 (2021).
Ding, L. et al. Antigen presentation by clonally diverse CXCR5+ B cells to CD4 and CD8 T cells is associated with durable response to immune checkpoint inhibitors. Front. Immunol. 14, 1176994 (2023).
Johansson-Percival, A. et al. De novo induction of intratumoral lymphoid structures and vessel normalization enhances immunotherapy in resistant tumors. Nat. Immunol. 18, 1207–1217 (2017).
Jin, Y. et al. Different syngeneic tumors show distinctive intrinsic tumor-immunity and mechanisms of actions (MOA) of anti-PD-1 treatment. Sci. Rep. 12, 3278 (2022).
Lee, H. et al. Integrated molecular and immunophenotypic analysis of NK cells in anti-PD-1 treated metastatic melanoma patients. Oncoimmunology 8, e1537581 (2019).
Sade-Feldman, M. et al. Resistance to checkpoint blockade therapy through inactivation of antigen presentation. Nat. Commun. 8, 1136 (2017).
Campbell, K. M. et al. Prior anti-CTLA-4 therapy impacts molecular characteristics associated with anti-PD-1 response in advanced melanoma. Cancer Cell 41, 791–806.e4 (2023).
Li, J. et al. Remodeling of the immune and stromal cell compartment by PD-1 blockade in mismatch repair-deficient colorectal cancer. Cancer Cell 41, 1152–1169.e7 (2023).
Jenkins, L. et al. Cancer-associated fibroblasts suppress CD8+ T-cell infiltration and confer resistance to immune-checkpoint blockade. Cancer Res. 82, 2904–2917 (2022).
Sivapalan, L. et al. Liquid biopsy approaches to capture tumor evolution and clinical outcomes during cancer immunotherapy. J. Immunother. Cancer 11, e005924 (2023).
Murray, J. C. et al. Elucidating the heterogeneity of immunotherapy response and immune-related toxicities by longitudinal ctDNA and immune cell compartment tracking in lung cancer. Clin. Cancer Res. 30, 389–403 (2023).
Anagnostou, V. et al. Dynamics of tumor and immune responses during immune checkpoint blockade in non-small cell lung cancer. Cancer Res. 79, 1214–1225 (2019).
Anagnostou, V. et al. ctDNA response after pembrolizumab in non-small cell lung cancer: phase 2 adaptive trial results. Nat. Med. 29, 2259–2569 (2023).
Kelly, R. J. et al. Neoadjuvant nivolumab or nivolumab plus LAG-3 inhibitor relatlimab in resectable esophageal/gastroesophageal junction cancer: a phase 1b trial and ctDNA analyses. Nat. Med. 30, 1023–1034 (2024).
Anagnostou, V. et al. Multimodal genomic features predict outcome of immune checkpoint blockade in non-small-cell lung cancer. Nat. Cancer 1, 99–111 (2020).
McGranahan, N. et al. Allele-specific HLA loss and immune escape in lung cancer evolution. Cell 171, 1259–1271.e11 (2017).
Peng, W. et al. Loss of PTEN promotes resistance to T cell-mediated immunotherapy. Cancer Discov. 6, 202–216 (2016).
Shin, D. S. et al. Primary resistance to PD-1 blockade mediated by JAK1/2 mutations. Cancer Discov. 7, 188–201 (2017).
Skoulidis, F. et al. STK11/LKB1 mutations and PD-1 inhibitor resistance in KRAS-mutant lung adenocarcinoma. Cancer Discov. 8, 822–835 (2018).
Spranger, S. & Gajewski, T. F. Impact of oncogenic pathways on evasion of antitumour immune responses. Nat. Rev. Cancer 18, 139–147 (2018).
Le, D. T. et al. Mismatch repair deficiency predicts response of solid tumors to PD-1 blockade. Science 357, 409–413 (2017).
Akbay, E. A. et al. Activation of the PD-1 pathway contributes to immune escape in EGFR-driven lung tumors. Cancer Discov. 3, 1355–1363 (2013).
Anagnostou, V. et al. Evolution of neoantigen landscape during immune checkpoint blockade in non-small cell lung cancer. Cancer Discov. 7, 264–276 (2017).
Niknafs, N. et al. Persistent mutation burden drives sustained anti-tumor immune responses. Nat. Med. 29, 440–449 (2023).
McGranahan, N. et al. Clonal neoantigens elicit T cell immunoreactivity and sensitivity to immune checkpoint blockade. Science 351, 1463–1469 (2016).
Gough, M. J. et al. Adjuvant therapy with agonistic antibodies to CD134 (OX40) increases local control after surgical or radiation therapy of cancer in mice. J. Immunother. 33, 798–809 (2010).
Pittman, K. & Kubes, P. Damage-associated molecular patterns control neutrophil recruitment. J. Innate Immun. 5, 315–323 (2013).
Singel, K. L. & Segal, B. H. Neutrophils in the tumor microenvironment: trying to heal the wound that cannot heal. Immunol. Rev. 273, 329–343 (2016).
Gregorio, J. et al. Plasmacytoid dendritic cells sense skin injury and promote wound healing through type I interferons. J. Exp. Med. 207, 2921–2930 (2010).
He, L. & Marneros, A. G. Macrophages are essential for the early wound healing response and the formation of a fibrovascular scar. Am. J. Pathol. 182, 2407–2417 (2013).
Muller, E. et al. Toll-like receptor ligands and interferon-γ synergize for induction of antitumor M1 macrophages. Front. Immunol. 8, 1383 (2017).
Wang, X. et al. T lymphocytes attenuate dermal scarring by regulating inflammation, neovascularization, and extracellular matrix remodeling. Adv. Wound Care 8, 527–537 (2019).
Pena, O. A. & Martin, P. Cellular and molecular mechanisms of skin wound healing. Nat. Rev. Mol. Cell Biol. https://doi.org/10.1038/s41580-024-00715-1 (2024).
Ploeger, D. T. et al. Cell plasticity in wound healing: paracrine factors of M1/M2 polarized macrophages influence the phenotypical state of dermal fibroblasts. Cell Commun. Signal. 11, 29 (2013).
Lucas, T. et al. Differential roles of macrophages in diverse phases of skin repair. J. Immunol. 184, 3964–3977 (2010).
Boothby, I. C., Cohen, J. N. & Rosenblum, M. D. Regulatory T cells in skin injury: at the crossroads of tolerance and tissue repair. Sci. Immunol. 5, eaaz9631 (2020).
Hu, K. H. et al. Transcriptional space-time mapping identifies concerted immune and stromal cell patterns and gene programs in wound healing and cancer. Cell Stem Cell 30, 885–903.e10 (2023).
Nosbaum, A. et al. Cutting edge: regulatory T cells facilitate cutaneous wound healing. J. Immunol. 196, 2010–2014 (2016).
Donati, G. et al. Wounding induces dedifferentiation of epidermal Gata6+ cells and acquisition of stem cell properties. Nat. Cell Biol. 19, 603–613 (2017).
Yoon, H. et al. TGF-β1-mediated transition of resident fibroblasts to cancer-associated fibroblasts promotes cancer metastasis in gastrointestinal stromal tumor. Oncogenesis 10, 13 (2021).
Chakravarthy, A., Khan, L., Bensler, N. P., Bose, P. & De Carvalho, D. D. TGF-β-associated extracellular matrix genes link cancer-associated fibroblasts to immune evasion and immunotherapy failure. Nat. Commun. 9, 4692 (2018).
Patel, S. P. et al. Neoadjuvant–adjuvant or adjuvant-only pembrolizumab in advanced melanoma. N. Engl. J. Med. 388, 813–823 (2023).
Cloughesy, T. F. et al. Neoadjuvant anti-PD-1 immunotherapy promotes a survival benefit with intratumoral and systemic immune responses in recurrent glioblastoma. Nat. Med. 25, 477–486 (2019).
van Dijk, N. et al. Preoperative ipilimumab plus nivolumab in locoregionally advanced urothelial cancer: the NABUCCO trial. Nat. Med. 26, 1839–1844 (2020).
Chalabi, M. et al. Neoadjuvant immunotherapy leads to pathological responses in MMR-proficient and MMR-deficient early-stage colon cancers. Nat. Med. 26, 566–576 (2020).
Vos, J. L. et al. Neoadjuvant immunotherapy with nivolumab and ipilimumab induces major pathological responses in patients with head and neck squamous cell carcinoma. Nat. Commun. 12, 7348 (2021).
Cascone, T. et al. Neoadjuvant nivolumab or nivolumab plus ipilimumab in operable non-small cell lung cancer: the phase 2 randomized NEOSTAR trial. Nat. Med. 27, 504–514 (2021).
Blank, C. U. et al. Neoadjuvant versus adjuvant ipilimumab plus nivolumab in macroscopic stage III melanoma. Nat. Med. 24, 1655–1661 (2018).
Topalian, S. L., Taube, J. M. & Pardoll, D. M. Neoadjuvant checkpoint blockade for cancer immunotherapy. Science 367, eaax0182 (2020).
Tilsed, C. M., Fisher, S. A., Nowak, A. K., Lake, R. A. & Lesterhuis, W. J. Cancer chemotherapy: insights into cellular and tumor microenvironmental mechanisms of action. Front. Oncol. 12, 960317 (2022).
Paz-Ares, L. et al. Pembrolizumab plus chemotherapy for squamous non-small-cell lung cancer. N. Engl. J. Med. 379, 2040–2051 (2018).
Eskander, R. N. et al. Pembrolizumab plus chemotherapy in advanced endometrial cancer. N. Engl. J. Med. 388, 2159–2170 (2023).
Colombo, N. et al. Pembrolizumab for persistent, recurrent, or metastatic cervical cancer. N. Engl. J. Med. 385, 1856–1867 (2021).
Schmid, P. et al. Event-free survival with pembrolizumab in early triple-negative breast cancer. N. Engl. J. Med. 386, 556–567 (2022).
Janjigian, Y. Y. et al. First-line nivolumab plus chemotherapy versus chemotherapy alone for advanced gastric, gastro-oesophageal junction, and oesophageal adenocarcinoma (CheckMate 649): a randomised, open-label, phase 3 trial. Lancet 398, 27–40 (2021).
Park, Y. H. et al. Chemotherapy induces dynamic immune responses in breast cancers that impact treatment outcome. Nat. Commun. 11, 6175 (2020).
Hui, Z. et al. PD-1 blockade potentiates neoadjuvant chemotherapy in NSCLC via increasing CD127+ and KLRG1+ CD8 T cells. NPJ Precis. Oncol. 7, 48 (2023).
Schumacher, T. N. & Thommen, D. S. Tertiary lymphoid structures in cancer. Science 375, eabf9419 (2022).
Schmidt, E. V. et al. Assessment of clinical activity of PD-1 checkpoint inhibitor combination therapies reported in clinical trials. JAMA Netw. Open 3, e1920833 (2020).
Reck, M. et al. First-line nivolumab plus ipilimumab with two cycles of chemotherapy versus chemotherapy alone (four cycles) in advanced non-small-cell lung cancer: CheckMate 9LA 2-year update. ESMO Open 6, 100273 (2021).
Yabuuchi, H. et al. Non-small cell lung cancer: detection of early response to chemotherapy by using contrast-enhanced dynamic and diffusion-weighted MR imaging. Radiology 261, 598–604 (2011).
Principe, N. et al. Comprehensive testing of chemotherapy and immune checkpoint blockade in preclinical cancer models identifies additive combinations. Front. Immunol. 13, 872295 (2022).
Weichselbaum, R. R., Liang, H., Deng, L. & Fu, Y. X. Radiotherapy and immunotherapy: a beneficial liaison? Nat. Rev. Clin. Oncol. 14, 365–379 (2017).
Deng, L. et al. STING-dependent cytosolic DNA sensing promotes radiation-induced type I interferon-dependent antitumor immunity in immunogenic tumors. Immunity 41, 843–852 (2014).
Herrera, F. G. et al. Low-dose radiotherapy reverses tumor immune desertification and resistance to immunotherapy. Cancer Discov. 12, 108–133 (2022).
Burnette, B. C. et al. The efficacy of radiotherapy relies upon induction of type I interferon-dependent innate and adaptive immunity. Cancer Res. 71, 2488–2496 (2011).
Klopp, A. H. et al. Tumor irradiation increases the recruitment of circulating mesenchymal stem cells into the tumor microenvironment. Cancer Res. 67, 11687–11695 (2007).
Yang, L., Pang, Y. & Moses, H. L. TGF-β and immune cells: an important regulatory axis in the tumor microenvironment and progression. Trends Immunol. 31, 220–227 (2010).
Seifert, L. et al. Radiation therapy induces macrophages to suppress T-cell responses against pancreatic tumors in mice. Gastroenterology 150, 1659–1672.e5 (2016).
Wu, Q. et al. Macrophage biology plays a central role during ionizing radiation-elicited tumor response. Biomed. J. 40, 200–211 (2017).
Shaverdian, N. et al. Previous radiotherapy and the clinical activity and toxicity of pembrolizumab in the treatment of non-small-cell lung cancer: a secondary analysis of the KEYNOTE-001 phase 1 trial. Lancet Oncol. 18, 895–903 (2017).
Antonia, S. J. et al. Durvalumab after chemoradiotherapy in stage III non-small-cell lung cancer. N. Engl. J. Med. 377, 1919–1929 (2017).
Faivre-Finn, C. et al. Impact of prior chemoradiotherapy-related variables on outcomes with durvalumab in unresectable stage III NSCLC (PACIFIC). Lung Cancer 151, 30–38 (2021).
Yang, Y. et al. Efficacy and safety of combined brain radiotherapy and immunotherapy in non-small-cell lung cancer with brain metastases: a systematic review and meta-analysis. Clin. Lung Cancer 23, 95–107 (2022).
Rodriguez-Ruiz, M. E. et al. Abscopal effects of radiotherapy are enhanced by combined immunostimulatory mabs and are dependent on CD8 T cells and crosspriming. Cancer Res. 76, 5994–6005 (2016).
Rompre-Brodeur, A. et al. PD-1/PD-L1 immune checkpoint inhibition with radiation in bladder cancer: in situ and abscopal effects. Mol. Cancer Ther. 19, 211–220 (2020).
Dovedi, S. J. et al. Acquired resistance to fractionated radiotherapy can be overcome by concurrent PD-L1 blockade. Cancer Res. 74, 5458–5468 (2014).
Young, K. H. et al. Optimizing timing of immunotherapy improves control of tumors by hypofractionated radiation therapy. PLoS ONE 11, e0157164 (2016).
Frederick, D. T. et al. BRAF inhibition is associated with enhanced melanoma antigen expression and a more favorable tumor microenvironment in patients with metastatic melanoma. Clin. Cancer Res. 19, 1225–1231 (2013).
Wilmott, J. S. et al. Selective BRAF inhibitors induce marked T-cell infiltration into human metastatic melanoma. Clin. Cancer Res. 18, 1386–1394 (2012).
Steinberg, S. M. et al. BRAF inhibition alleviates immune suppression in murine autochthonous melanoma. Cancer Immunol. Res. 2, 1044–1050 (2014).
Schilling, B. et al. Vemurafenib reverses immunosuppression by myeloid derived suppressor cells. Int. J. Cancer 133, 1653–1663 (2013).
Arthur, J. S. & Ley, S. C. Mitogen-activated protein kinases in innate immunity. Nat. Rev. Immunol. 13, 679–692 (2013).
Tel, J. et al. Preclinical exploration of combining plasmacytoid and myeloid dendritic cell vaccination with BRAF inhibition. J. Transl. Med. 14, 88 (2016).
Vella, L. J. et al. MEK inhibition, alone or in combination with BRAF inhibition, affects multiple functions of isolated normal human lymphocytes and dendritic cells. Cancer Immunol. Res. 2, 351–360 (2014).
Deken, M. A. et al. Targeting the MAPK and PI3K pathways in combination with PD1 blockade in melanoma. Oncoimmunology 5, e1238557 (2016).
Hugo, W. et al. Non-genomic and immune evolution of melanoma acquiring MAPKi resistance. Cell 162, 1271–1285 (2015).
Pieper, N. et al. Evolution of melanoma cross-resistance to CD8+ T cells and MAPK inhibition in the course of BRAFi treatment. Oncoimmunology 7, e1450127 (2018).
Haas, L. et al. Acquired resistance to anti-MAPK targeted therapy confers an immune-evasive tumor microenvironment and cross-resistance to immunotherapy in melanoma. Nat. Cancer 2, 693–708 (2021).
Kumagai, S., Koyama, S. & Nishikawa, H. Antitumour immunity regulated by aberrant ERBB family signalling. Nat. Rev. Cancer 21, 181–197 (2021).
Maynard, A. et al. Therapy-induced evolution of human lung cancer revealed by single-cell RNA sequencing. Cell 182, 1232–1251.e22 (2020).
Jia, Y. et al. EGFR-targeted therapy alters the tumor microenvironment in EGFR-driven lung tumors: implications for combination therapies. Int. J. Cancer 145, 1432–1444 (2019).
Venugopalan, A. et al. EGFR-targeted therapy results in dramatic early lung tumor regression accompanied by imaging response and immune infiltration in EGFR mutant transgenic mouse models. Oncotarget 7, 54137–54156 (2016).
Isomoto, K. et al. Impact of EGFR-TKI treatment on the tumor immune microenvironment in EGFR mutation-positive non-small cell lung cancer. Clin. Cancer Res. 26, 2037–2046 (2020).
Patel, S. A. et al. Molecular mechanisms and future implications of VEGF/VEGFR in cancer therapy. Clin. Cancer Res. 29, 30–39 (2023).
Adachi, Y. et al. Inhibition of FGFR reactivates IFNγ signaling in tumor cells to enhance the combined antitumor activity of lenvatinib with anti-PD-1 antibodies. Cancer Res. 82, 292–306 (2022).
Kato, Y. et al. Lenvatinib plus anti-PD-1 antibody combination treatment activates CD8+ T cells through reduction of tumor-associated macrophage and activation of the interferon pathway. PLoS ONE 14, e0212513 (2019).
Mei, Z. et al. Lenvatinib enhances antitumor immunity by promoting the infiltration of TCF1+ CD8+ T cells in HCC via blocking VEGFR2. Cancer Sci. 114, 1284–1296 (2023).
Finke, J. H. et al. Sunitinib reverses type-1 immune suppression and decreases T-regulatory cells in renal cell carcinoma patients. Clin. Cancer Res. 14, 6674–6682 (2008).
Long, G. V. et al. NeoTrio: randomized trial of neoadjuvant (NAT) pembrolizumab (Pembro) alone, in sequence (SEQ) with, or concurrent (CON) with dabrafenib plus trametinib (D+T) in resectable BRAF-mutant stage III melanoma to determine optimal combination of therapy. J. Clin. Oncol. 40, 9503–9503 (2022).
Ascierto, P. A. et al. Sequencing of ipilimumab plus nivolumab and encorafenib plus binimetinib for untreated BRAF-mutated metastatic melanoma (SECOMBIT): a randomized, three-arm, open-label phase II trial. J. Clin. Oncol. 41, 212–221 (2023).
Yang, J. C.-H. et al. Osimertinib plus durvalumab versus osimertinib monotherapy in EGFR T790M–positive NSCLC following previous EGFR TKI therapy: CAURAL brief report. J. Thorac. Oncol. 14, 933–939 (2019).
Motzer, R. J. et al. Avelumab plus axitinib versus sunitinib for advanced renal-cell carcinoma. N. Engl. J. Med. 380, 1103–1115 (2019).
Choueiri, T. K. et al. Updated efficacy results from the JAVELIN Renal 101 trial: first-line avelumab plus axitinib versus sunitinib in patients with advanced renal cell carcinoma. Ann. Oncol. 31, 1030–1039 (2020).
Singh, K. et al. Correcting the drug development paradigm for glioblastoma requires serial tissue sampling. Nat. Med. 29, 2402–2405 (2023).
Fourie Zirkelbach, J. et al. Improving dose-optimization processes used in oncology drug development to minimize toxicity and maximize benefit to patients. J. Clin. Oncol. 40, 3489–3500 (2022).
Janssens, G. O. et al. Recommendations for the organisation of care in paediatric radiation oncology across Europe: a SIOPE-ESTRO-PROS-CCI-Europe collaborative project in the framework of the JARC. Eur. J. Cancer 114, 47–54 (2019).
Siegel, S. E. et al. Pediatric-inspired treatment regimens for adolescents and young adults with Philadelphia chromosome-negative acute lymphoblastic leukemia: a review. JAMA Oncol. 4, 725–734 (2018).
Rwandamuriye, F. X. et al. A surgically optimized intraoperative poly(I:C)-releasing hydrogel prevents cancer recurrence. Cell Rep. Med. 4, 101113 (2023).
Park, C. G. et al. Extended release of perioperative immunotherapy prevents tumor recurrence and eliminates metastases. Sci. Transl. Med. 10, eaar1916 (2018).
Jin, S. M. et al. A nanoadjuvant that dynamically coordinates innate immune stimuli activation enhances cancer immunotherapy and reduces immune cell exhaustion. Nat. Nanotechnol. 18, 390–402 (2023).
Wang, H. & Mooney, D. J. Biomaterial-assisted targeted modulation of immune cells izcancer treatment. Nat. Mater. 17, 761–772 (2018).
Reijers, I. L. M. et al. IFN-γ signature enables selection of neoadjuvant treatment in patients with stage III melanoma. J. Exp. Med. 220, e20221952 (2023).
Huang, A. C. et al. A single dose of neoadjuvant PD-1 blockade predicts clinical outcomes in resectable melanoma. Nat. Med. 25, 454–461 (2019).
Tumeh, P. C. et al. PD-1 blockade induces responses by inhibiting adaptive immune resistance. Nature 515, 568–571 (2014).
Abril-Rodriguez, G. et al. PAK4 inhibition improves PD-1 blockade immunotherapy. Nat. Cancer 1, 46–58 (2020).
Cabrita, R. et al. Tertiary lymphoid structures improve immunotherapy and survival in melanoma. Nature 577, 561–565 (2020).
Liu, D. et al. Integrative molecular and clinical modeling of clinical outcomes to PD1 blockade in patients with metastatic melanoma. Nat. Med. 25, 1916–1927 (2019).
Van Allen, E. M. et al. Genomic correlates of response to CTLA-4 blockade in metastatic melanoma. Science 350, 207–211 (2015).
Wolchok, J. D. et al. Overall survival with combined nivolumab and ipilimumab in advanced melanoma. N. Engl. J. Med. 377, 1345–1356 (2017).
Weber, J. S. et al. Sequential administration of nivolumab and ipilimumab with a planned switch in patients with advanced melanoma (CheckMate 064): an open-label, randomised, phase 2 trial. Lancet Oncol. 17, 943–955 (2016).
Li, S. et al. Neoadjuvant therapy with immune checkpoint blockade, antiangiogenesis, and chemotherapy for locally advanced gastric cancer. Nat. Commun. 14, 8 (2023).
Lee, S. E. et al. Immunologic predictors for clinical responses during immune checkpoint blockade in patients with myelodysplastic syndromes. Clin. Cancer Res. 29, 1938–1951 (2023).
Liu, B. et al. Temporal single-cell tracing reveals clonal revival and expansion of precursor exhausted T cells during anti-PD-1 therapy in lung cancer. Nat. Cancer 3, 108–121 (2022).
Bi, K. et al. Tumor and immune reprogramming during immunotherapy in advanced renal cell carcinoma. Cancer Cell 39, 649–661 e645 (2021).
Krishna, C. et al. Single-cell sequencing links multiregional immune landscapes and tissue-resident T cells in ccRCC to tumor topology and therapy efficacy. Cancer Cell 39, 662–677.e6 (2021).
Yofe, I. et al. Anti-CTLA-4 antibodies drive myeloid activation and reprogram the tumor microenvironment through FcγR engagement and type I interferon signaling. Nat. Cancer 3, 1336–1350 (2022).
Sitnikova, S. I. et al. Novel non-terminal tumor sampling procedure using fine needle aspiration supports immuno-oncology biomarker discovery in preclinical mouse models. J. Immunother. Cancer 9, e002894 (2021).
Sugiyarto, G. et al. Reactivation of low avidity tumor-specific CD8+ T cells associates with immunotherapeutic efficacy of anti-PD-1. J. Immunother. Cancer 11, e007114 (2023).
Kurtulus, S. et al. Checkpoint blockade immunotherapy induces dynamic changes in PD-1−CD8+ tumor-infiltrating T cells. Immunity 50, 181–194.e6 (2019).
Menzies, A. M. et al. Pathological response and survival with neoadjuvant therapy in melanoma: a pooled analysis from the International Neoadjuvant Melanoma Consortium (INMC). Nat. Med. 27, 301–309 (2021).
Rozeman, E. A. et al. Identification of the optimal combination dosing schedule of neoadjuvant ipilimumab plus nivolumab in macroscopic stage III melanoma (OpACIN-neo): a multicentre, phase 2, randomised, controlled trial. Lancet Oncol. 20, 948–960 (2019).
Versluis, J. M. et al. Survival update of neoadjuvant ipilimumab plus nivolumab in macroscopic stage III melanoma in the OpACIN and OpACIN-neo trials. Ann. Oncol. 34, 420–430 (2023).
Reijers, I. L. M. et al. Personalized response-directed surgery and adjuvant therapy after neoadjuvant ipilimumab and nivolumab in high-risk stage III melanoma: the PRADO trial. Nat. Med. 28, 1178–1188 (2022).
Nabet, B. Y. et al. Noninvasive early identification of therapeutic benefit from immune checkpoint inhibition. Cell 183, 363–376.e13 (2020).
Georgiev, P. et al. Reverse translating molecular determinants of anti-programmed death 1 immunotherapy response in mouse syngeneic tumor models. Mol. Cancer Ther. 21, 427–439 (2022).
Mosely, S. I. et al. Rational selection of syngeneic preclinical tumor models for immunotherapeutic drug discovery. Cancer Immunol. Res. 5, 29–41 (2017).
Mantovani, A., Allavena, P., Marchesi, F. & Garlanda, C. Macrophages as tools and targets in cancer therapy. Nat. Rev. Drug Discov. 21, 799–820 (2022).
Codarri Deak, L. et al. PD-1-cis IL-2R agonism yields better effectors from stem-like CD8+ T cells. Nature 610, 161–172 (2022).
Hashimoto, M. et al. PD-1 combination therapy with IL-2 modifies CD8+ T cell exhaustion program. Nature 610, 173–181 (2022).
Amaria, R. N. et al. Neoadjuvant systemic therapy in melanoma: recommendations of the International Neoadjuvant Melanoma Consortium. Lancet Oncol. 20, e378–e389 (2019).
Acknowledgements
G.V.L. is supported by a National Health and Medical Research Council (NHMRC) Investigator Grant (2021/GNT2007839) and by the University of Sydney Medical Foundation. W.J.L. is supported by an NHMRC Investigator Grant (2021/GNT1196605).
Author information
Authors and Affiliations
Contributions
All authors researched data for the article, contributed substantially to the discussion of the content, wrote the article and reviewed the manuscript before submission.
Corresponding author
Ethics declarations
Competing Interests
R.M.Z. is an inventor on patent applications (WO/2019/178650A1 and PCT/AU2023/051218) related to ICT treatment combinations. V.A. receives research funding to Johns Hopkins University from AstraZeneca and Personal Genome Diagnostics, has received research funding to Johns Hopkins University from Bristol-Myers Squibb and Delfi Diagnostics in the past 5 years, is an advisory board member for AstraZeneca and Neogenomics and receives honoraria from Foundation Medicine and Personal Genome Diagnostics. V.A. is an inventor on patent applications (63/276,525, 17/779,936, 16/312,152, 16/341,862, 17/047,006 and 17/598,690) submitted by Johns Hopkins University related to cancer genomic analyses, ctDNA therapeutic response monitoring and immunogenomic features of response to immunotherapy that have been licensed to one or more entities. Under the terms of these license agreements, the university and inventors are entitled to fees and royalty distributions. I.P.S. had travel support by BMS and MSD, speaker fee by Pierre Fabre, Roche, BMS and MSD and has served as consultant on advisory boards from MSD. G.V.L. is consultant adviser for Agenus, Amgen, Array Biopharma, AstraZeneca, Bayer, BioNTech, Boehringer Ingelheim, Bristol Myers Squibb, Evaxion, Hexal AG (Sandoz Company), Highlight Therapeutics S.L., IOBiotech, Immunocore, Innovent Biologics USA, Merck Sharpe & Dohme, Novartis, PHMR Ltd, Pierre Fabre, Regeneron, Scancell and SkylineDX B.V. W.J.L. is consultant adviser for Douglas Pharmaceuticals, has received research funding from Douglas Pharmaceuticals, AstraZeneca and Axelia Oncology. W.J.L. is a founder and director of Setonix Pharmaceuticals and is inventor on patent applications (WO/2016/015095, WO/2019/178650A1 and PCT/AU2023/051218) related to ICT treatment combinations.
Peer review
Peer review information
Nature Reviews Cancer thanks the anonymous reviewer(s) for their contribution to the peer review of this work.
Additional information
Publisher’s note Springer Nature remains neutral with regard to jurisdictional claims in published maps and institutional affiliations.
Supplementary information
Glossary
- Adjuvant treatment
-
A treatment given after surgery to reduce the chance of cancer recurrence.
- Circulating tumour DNA
-
(ctDNA). Circulating tumour-derived fragmented DNA that can be used to assess minimal residual disease or capture intratumoural and temporal cancer heterogeneity.
- Clonotype richness
-
A measure of the number of T cell clones with a unique T cell receptor, wherein a higher clonotype richness indicates a wider array of T cell receptors within the T cell population.
- Co-stimulatory molecules
-
A cell surface protein that provides activation signals alongside primary antigen recognition to fully activate T cells.
- Effector memory T cell
-
A subset of memory T cells with effector activity.
- Fractionated doses
-
A delivery method of radiation to a site in which the total prescribed dose is divided into smaller doses over a period of time.
- Immune dynamics
-
Series of immune-mediated biological events that occur in a sequence over time.
- Myeloid-derived suppressor cell
-
(MDSC). A myeloid cell that arises under pathological conditions, defined by its T cell immunosuppressive functions.
- Neoadjuvant treatment
-
A treatment given (partially or completely) before surgery to increase the likelihood of complete surgical resection and to reduce the chance of cancer recurrence.
- Progenitor exhausted CD8+ T cells
-
(Tpex). Self-renewing CD8+ T cells with memory characteristics and high PD1 expression that are defined by the expression of the transcription factors TCF1 (encoded by the gene TCF7) and MYB, which replenish terminally exhausted effector cells.
- Resident memory T cell
-
A subset of memory T cells that persist in tissue in which they were locally activated.
- Terminally exhausted T cells
-
(Tex). Tpex cells give rise to Tex cells marked by CD38, CD39, PD1 and TIM3, which have diminished responsiveness to ICT, yet are the dominant phenotype of tumour antigen-specific T cells.
- Tertiary lymphoid structures
-
Formations of ectopic lymphoid organs within tumours that consist of B cells, T cells and supporting dendritic cells.
- Tumour-associated macrophages
-
A monocyte or macrophage-derived cells expressing macrophage markers, which can have a pro-inflammatory phenotype with antitumour functionality or an anti-inflammatory phenotype with tumour promoting effects.
- Tumour microenvironment
-
(TME). The immediate environment of a tumour that includes blood vessels, immune cells, signalling molecules, fibroblasts and extracellular matrix.
Rights and permissions
Springer Nature or its licensor (e.g. a society or other partner) holds exclusive rights to this article under a publishing agreement with the author(s) or other rightsholder(s); author self-archiving of the accepted manuscript version of this article is solely governed by the terms of such publishing agreement and applicable law.
About this article
Cite this article
Zemek, R.M., Anagnostou, V., Pires da Silva, I. et al. Exploiting temporal aspects of cancer immunotherapy. Nat Rev Cancer 24, 480–497 (2024). https://doi.org/10.1038/s41568-024-00699-2
Accepted:
Published:
Issue Date:
DOI: https://doi.org/10.1038/s41568-024-00699-2